Science
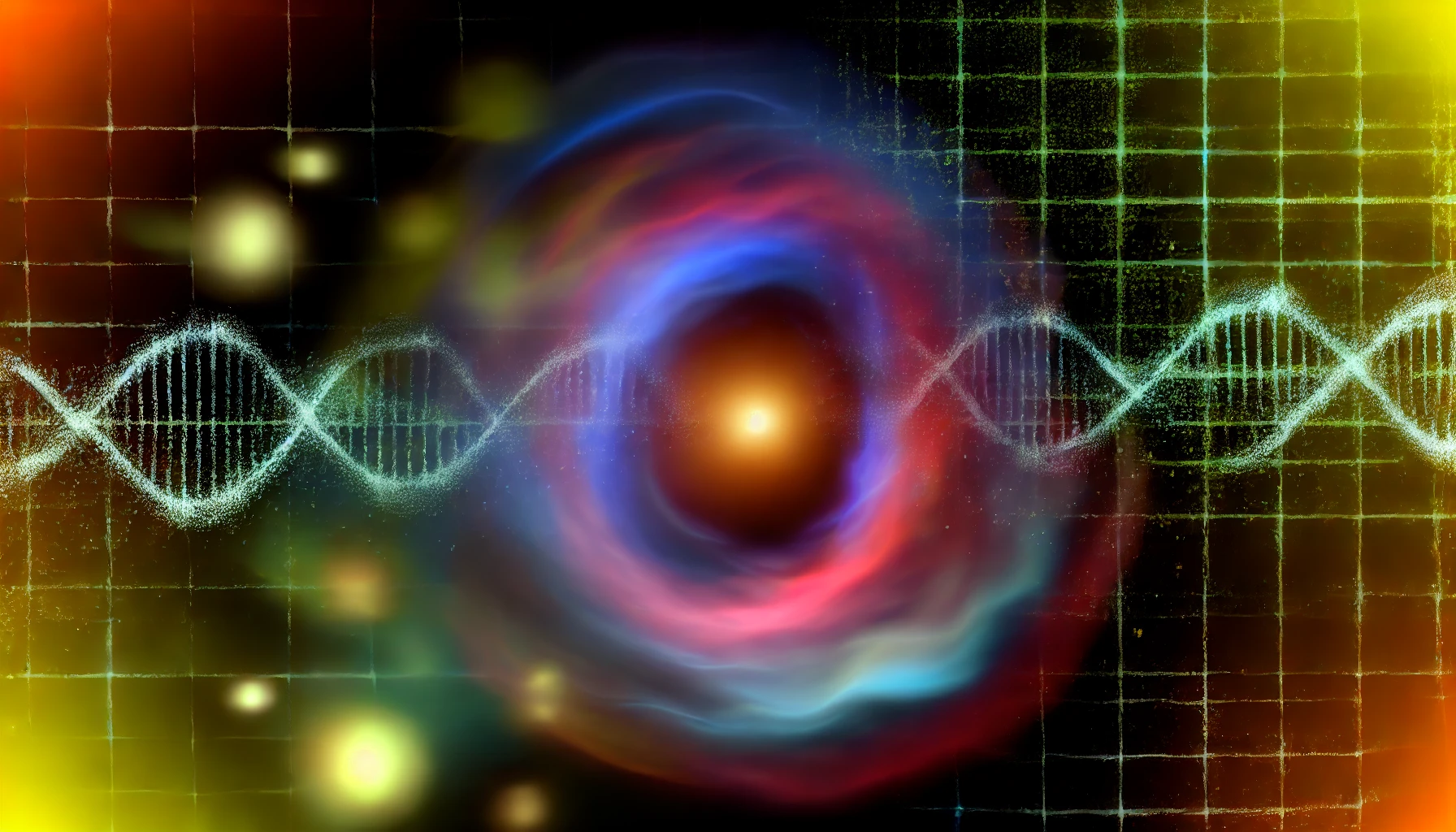
The Evolution of the Universe: From Pre-Cosmic Conditions to the Present
(work in progress)
The evolution of the universe is a profound narrative stretching back almost 14 billion years. From an initial state of extreme density and energy, the universe has undergone a series of critical transformations to become the vast cosmic web of galaxies, stars, and planets we observe today. Each stage of this transformation has played an essential role in shaping the cosmos. A thorough understanding of these stages enables us to reconstruct the past, interpret the present, and envision the future trajectory of the universe. From pre-Big Bang conditions to the current era of accelerated expansion, the universe has evolved through numerous significant events, each contributing to its complexity.
Hypotheses on What Might Have Preceded Cosmic Inflation
The nature of the universe prior to the onset of cosmic inflation is a major area of theoretical exploration. Several hypotheses attempt to elucidate this enigmatic period preceding inflation.
One prominent hypothesis suggests that the universe began in a quantum state, often referred to as a "false vacuum," characterized by high potential energy and dominated by quantum fluctuations (Guth, 1981). In this scenario, the universe existed in an unstable energy state, and the decay of this false vacuum led to a rapid period of inflation. This inflationary model provides a mechanism by which an almost featureless quantum state could evolve into the structured universe we observe today.
Another hypothesis posits that the universe follows a cyclic model, in which it undergoes perpetual cycles of contraction and expansion (Steinhardt & Turok, 2002). According to this model, our universe may be one phase in an eternal sequence of cosmic cycles, where each collapse leads to a subsequent expansion, effectively resulting in a perpetual regeneration of the cosmos.
In some other models, cosmic inflation was triggered by a fluctuation within a pre-existing quantum vacuum. Quantum uncertainties in this vacuum might have caused a rapid expansion, igniting the universe into the inflationary phase that eventually led to the Big Bang (Vilenkin, 1983).
Cosmic Inflation (10-36 till 10-32 seconds after the big bang)
Cosmic inflation refers to an extremely brief yet exponential expansion of space that occurred within the first fractions of a second after the Big Bang. During this period, the universe expanded from subatomic scales to a macroscopic size, effectively smoothing out inhomogeneities and accounting for the observable uniformity of the cosmos (Linde, 1982). The driving force behind this rapid expansion was the inflaton field, which stored vast amounts of potential energy and fueled the exponential growth.
The idea of cosmic inflation emerged to address limitations inherent in the traditional Big Bang model. One of these issues was the horizon problem. The universe appears remarkably homogeneous on large scales, as demonstrated by the nearly uniform temperature of the cosmic microwave background (CMB) radiation, despite the fact that distant regions should not have been in causal contact given the finite speed of light and the age of the universe. Inflation offers a solution by proposing a period of exponential expansion, effectively allowing regions now separated by vast distances to have once been causally connected.
Another fundamental issue was the flatness problem. Observations show that the universe is nearly spatially flat, requiring precise fine-tuning of the density parameter, Ω, during its early evolution. Inflation naturally drives the universe towards a flat geometry, much like how the surface of an inflating balloon appears flatter as it expands.
Finally, the monopole problem stems from Grand Unified Theories (GUTs), which predict the formation of magnetic monopoles—hypothetical particles that are extremely massive and should have been produced in abundance during the early universe. However, no magnetic monopoles have been observed. The rapid inflationary expansion dilutes their concentration to near undetectable levels, resolving this apparent discrepancy.
The Mechanics of Inflation
The dynamics of inflation are governed by a scalar field, often called the inflaton, whose potential energy drives the exponential expansion. During inflation, the universe grew by a factor of at least 1026. This immense expansion causes quantum fluctuations in the inflaton field to stretch and become classical density perturbations, eventually seeding the formation of galaxies and large-scale structures.
A widely studied model of inflation involves a slow-roll approximation of the inflaton field, wherein the potential energy dominates over the kinetic energy, allowing the universe to expand rapidly. The slow-roll conditions are characterized by two dimensionless parameters, ε and η, which ensure that the potential is sufficiently flat for inflation to occur. The end of inflation is marked by a process called reheating, where the energy stored in the inflaton field is transferred to radiation and particles, transitioning the universe into the radiation-dominated phase described by the traditional Big Bang model.
Observational Evidence
Inflation has found strong support through its ability to predict and explain the properties of the cosmic microwave background radiation. Observations by the Wilkinson Microwave Anisotropy Probe (WMAP) and the Planck satellite have provided detailed measurements of the CMB, revealing tiny temperature anisotropies. These anisotropies are consistent with quantum fluctuations predicted by inflation, which were stretched to macroscopic scales and imprinted on the CMB. The nearly scale-invariant power spectrum of these fluctuations is a key prediction of inflation, and it matches observational data extremely well.
Moreover, inflation predicts the existence of primordial gravitational waves—ripples in spacetime generated during the inflationary epoch. These waves would leave an imprint in the form of B-mode polarization in the CMB. While direct detection of such primordial gravitational waves remains elusive, efforts like the BICEP2 experiment have provided tantalizing hints that continue to motivate ongoing research.
Challenges and Alternatives
Despite its successes, inflation is not without challenges. One significant issue is the lack of a definitive understanding of the inflaton's nature and its potential. Various models of inflation exist, ranging from chaotic inflation proposed by Andrei Linde to more recent ideas involving brane inflation and multiverse scenarios. The diversity of models has led some physicists to explore alternatives to inflation, such as bouncing cosmologies or the ekpyrotic model, which posits that the universe underwent a contraction phase before expanding.
Another major challenge is the issue of eternal inflation. Some models predict that inflation never completely ends but rather continues in some regions of spacetime, leading to a multiverse where different regions stop inflating at different times, each potentially with different physical constants and properties. This raises profound questions about the nature of our universe and the role of anthropic reasoning in understanding its initial conditions.
The Reheating Phase (from 10-32 seconds until potentially a few minutes)
The reheating phase is a fascinating but often underappreciated era in the history of the universe, representing a crucial bridge between the inflationary epoch and the Hot Big Bang. This phase was instrumental in transitioning the universe from a state dominated by the inflaton field to a hot, radiation-dominated state filled with particles and energy, setting the stage for the formation of galaxies, stars, and ultimately, life. Understanding reheating provides insight into the thermal history of the universe and the mechanisms that produced the fundamental particles and forces that govern the cosmos today.
The End of Inflation and the Onset of Reheating
Cosmic inflation, proposed by physicist Alan Guth in the early 1980s, was a period of rapid, exponential expansion that occurred within the first 10−36 to 10−32 seconds after the Big Bang. During this period, the universe expanded by at least 1026 times, smoothing out any initial irregularities and making the universe appear homogenous and isotropic on large scales. However, when inflation ended, the universe was left cold and almost devoid of conventional particles, with most of its energy locked in the inflaton field.
Reheating was the process by which the energy from the inflaton field was converted into radiation and particles, thereby 'reheating' the universe and populating it with the ingredients necessary for the subsequent stages of cosmic evolution. During this period, the oscillations of the inflaton field around the minimum of its potential caused it to decay into a variety of elementary particles, which then thermalized, establishing a hot, dense state characteristic of the Hot Big Bang model.
Mechanisms of Reheating
The process of reheating involved complex interactions between the inflaton field and other quantum fields. Two primary mechanisms contributed to reheating: perturbative decay and parametric resonance (also known as preheating).
-
Perturbative Decay: In this mechanism, the inflaton field decays slowly into other particles through quantum processes similar to radioactive decay. This gradual process leads to the production of a thermal bath of particles, although the efficiency of this mechanism depends on the coupling strength between the inflaton and the particles it produces.
-
Parametric Resonance (Preheating): Preheating was a more explosive process, wherein the inflaton's oscillations led to the rapid and efficient transfer of energy to specific quantum modes. This created bursts of particle production that contributed significantly to the thermalization of the universe. Preheating is often a highly non-linear process, involving complex interactions that may produce a wide variety of particles, including those not part of the Standard Model of particle physics.
The energy released during these processes reheated the universe to extremely high temperatures, which may have reached up to GeV, although the exact value depends on the specifics of the inflaton's potential and its interactions. This temperature set the initial conditions for the radiation-dominated era that followed.
The Importance of Reheating for the Universe's Evolution
The reheating phase was crucial for several reasons. First, it set the temperature of the universe at the onset of the Hot Big Bang, establishing the conditions under which primordial nucleosynthesis could later occur, ultimately leading to the formation of light elements such as hydrogen, helium, and trace amounts of lithium. Second, the energy conversion during reheating populated the universe with fundamental particles—quarks, leptons, gauge bosons—that would later coalesce into more complex structures.
Reheating also played an important role in the baryogenesis process, which is responsible for the matter-antimatter asymmetry observed in the universe today. For baryogenesis to occur, certain conditions outlined by Andrei Sakharov must be satisfied, including baryon number violation, CP (charge-parity) symmetry violation, and interactions out of thermal equilibrium. The reheating phase provided a unique environment where these conditions could potentially be met, thereby allowing for the slight excess of matter over antimatter that eventually formed stars, galaxies, and planets.
Electroweak Symmetry Breaking and the Formation of Forces
As the universe cooled during reheating, significant changes occurred that shaped the fundamental forces. The electroweak force—a unified combination of the electromagnetic and weak nuclear forces—began to split as the temperature dropped. This symmetry breaking was driven by the Higgs field, which acquired a non-zero vacuum expectation value, giving mass to the W and Z bosons, which mediate the weak force, while leaving the photon massless, thus preserving electromagnetic interactions.
This breaking of electroweak symmetry was a vital step in forming the four fundamental forces as we know them today: gravity, electromagnetism, the weak nuclear force, and the strong nuclear force. The interactions of the Higgs field during reheating ultimately contributed to the masses of fundamental particles, setting the stage for the emergence of complex atomic structures once the universe cooled further.
Transition to the Hot Big Bang Phase
By the end of the reheating period, the universe had transitioned into a radiation-dominated phase, characterized by a hot, dense plasma of photons, neutrinos, quarks, and leptons. The establishment of thermal equilibrium allowed the universe to continue expanding and cooling in a predictable manner, as described by the traditional Big Bang theory. During this time, quarks combined to form hadrons such as protons and neutrons in a process called hadronization.
As the universe cooled further, these protons and neutrons underwent primordial nucleosynthesis, forming the light nuclei that would eventually combine with electrons to create the first neutral atoms. This set the stage for the next key phase of the universe’s evolution—recombination—which allowed light to travel freely for the first time, leading to the formation of the cosmic microwave background (CMB), the relic radiation that we observe today as a snapshot of the universe approximately 380,000 years after the Big Bang.
Challenges and Open Questions
Despite its importance, many details of the reheating phase remain elusive. One of the significant challenges lies in identifying the exact nature of the inflaton field and understanding its couplings to other particles. The lack of direct observational evidence makes it challenging to determine the precise dynamics of reheating, although indirect evidence can be gathered by studying the effects on the CMB and large-scale structure.
Another open question is whether non-standard particles or dark matter could have been produced during reheating. If dark matter was generated during this phase, it would have significant implications for its abundance and properties. The reheating temperature also affects the spectrum of primordial gravitational waves, which, if detected, could provide invaluable insights into the processes that took place during this enigmatic period.
The Hot Big Bang Phase (from fractions of a second until 380,000 years)
The Hot Big Bang phase marks a pivotal era in the history of the universe, transitioning from the end of cosmic inflation to a highly dynamic state dominated by radiation, rapid expansion, and the formation of the first atomic nuclei. During this phase, the universe evolved from an extremely hot, dense plasma into a gradually cooling environment, setting the foundation for all subsequent cosmic structures, including galaxies, stars, and planets. This phase not only produced the observable universe's earliest light—captured today as the Cosmic Microwave Background (CMB)—but also led to the formation of fundamental particles and elements that underpin all matter as we know it. A detailed understanding of this period provides essential insights into the universe's origins, composition, and long-term evolution.
From Inflation to the Hot Big Bang: Reheating and Thermalization
The Hot Big Bang phase began after the inflationary epoch, which saw the universe expand exponentially over an infinitesimal period of time. Following the end of inflation, the universe underwent a phase known as reheating. During reheating, the energy stored in the inflaton field—the scalar field responsible for inflation—was converted into radiation and matter, effectively repopulating the universe with particles (Kofman, Linde, & Starobinsky, 1997). This process marked the start of the radiation-dominated era.
During reheating, the universe thermalized, meaning that particles interacted and exchanged energy until they reached a state of thermal equilibrium. The thermal bath established by this process reached incredibly high temperatures, potentially as high as 1015 GeV, depending on the specific model of inflation. The conditions present during reheating were instrumental in defining the early universe's properties, including its temperature, particle content, and density, and set the stage for the physical processes that would follow.
The Hot Plasma: Quark-Gluon Soup and Hadronization
In the immediate aftermath of reheating, the universe was filled with a hot, dense plasma of quarks, gluons, leptons, and photons. The temperature was so high that quarks could not combine into hadrons, and instead existed in a free state known as a quark-gluon plasma. This plasma phase persisted for a brief period until the universe cooled sufficiently, at which point hadronization occurred, leading quarks to bind together to form protons and neutrons (Gross, Wilczek, & Politzer, 1973).
Hadronization was a critical moment in the Hot Big Bang phase, as it marked the formation of the first stable particles capable of undergoing nuclear reactions. The emergence of protons and neutrons allowed for the conditions that led to the synthesis of light atomic nuclei during the subsequent epoch of primordial nucleosynthesis.
Primordial Nucleosynthesis: The Formation of Light Elements
One of the defining features of the Hot Big Bang phase was primordial nucleosynthesis, which occurred roughly three minutes after the Big Bang, when the universe had cooled to around 109K (Peebles, 1966). During this period, protons and neutrons collided to form the first atomic nuclei, resulting in the creation of light elements, primarily hydrogen, helium, and trace amounts of lithium and beryllium.
Primordial nucleosynthesis was governed by the strong nuclear force and the relative abundances of protons and neutrons. Because neutrons are slightly heavier than protons, they were less stable and began to decay. However, the rapid formation of helium nuclei, composed of two protons and two neutrons, helped preserve a significant number of neutrons. The final abundance of elements produced during primordial nucleosynthesis has been verified by observations of the light element ratios in the oldest, most pristine regions of the universe, providing strong evidence for the Hot Big Bang model (Alpher, Bethe, & Gamow, 1948).
The resulting element abundances from primordial nucleosynthesis were approximately 75% hydrogen, 25% helium by mass, with trace amounts of other light elements. This specific elemental composition would eventually play a critical role in the formation of stars and galaxies, as it determined the characteristics of the first generation of stellar objects.
Radiation-Dominated Expansion and Decoupling
Following nucleosynthesis, the universe remained in a radiation-dominated state, where radiation (primarily in the form of photons and neutrinos) provided the dominant contribution to the energy density of the cosmos. During this phase, the expansion of the universe was driven by the properties of relativistic particles, causing the scale factor to grow rapidly. The interactions between radiation and matter were intense, as photons continuously scattered off free electrons, preventing light from traveling any significant distance without being absorbed or scattered.
The universe remained opaque to radiation until approximately 380,000 years after the Big Bang, when it cooled to a temperature of about 3000 K. At this point, protons and electrons combined to form neutral hydrogen in a process known as recombination. The formation of neutral atoms drastically reduced the scattering of photons, allowing radiation to travel freely through space. This event marked the decoupling of matter and radiation, leading to the release of the Cosmic Microwave Background (CMB) (Penzias & Wilson, 1965; Planck Collaboration, 2018).
The CMB represents the oldest light in the universe, providing a snapshot of the conditions at the time of decoupling. The nearly uniform temperature of the CMB, along with its tiny fluctuations, provides crucial information about the density variations that eventually grew into the large-scale structure of galaxies and clusters observed today. The detailed measurements of the CMB by the Wilkinson Microwave Anisotropy Probe (WMAP) and the Planck satellite have provided deep insights into the early universe, including the geometry, composition, and rate of expansion of the cosmos.
Transition to Matter Domination and Structure Formation
After decoupling, the universe transitioned from a radiation-dominated phase to a matter-dominated phase, as the energy density of non-relativistic matter began to exceed that of radiation. During this transition, the initial density fluctuations in the matter distribution, which had been seeded by quantum fluctuations during inflation, began to grow under the influence of gravity (White & Rees, 1978).
Dark matter played a crucial role in this process. While baryonic matter (ordinary matter) interacted with radiation and was influenced by pressure forces, dark matter experienced no such interactions and began to clump together earlier, forming the gravitational wells that would later attract baryonic matter. These dark matter structures acted as the scaffolding for the formation of the first galaxies and clusters, leading to the cosmic web-like structure that characterizes the universe today.
Recombination and the Formation of Neutral Atoms
Following the Hot Big Bang phase, the universe entered a crucial period known as recombination, which marks a pivotal milestone in the cosmic timeline. Occurring roughly 380,000 years after the Big Bang, recombination set the stage for the universe to become transparent, allowing photons to travel freely through space. This transition ultimately led to the creation of the Cosmic Microwave Background (CMB), a relic radiation that provides a snapshot of the universe during this transformative period (Peebles, 1968; Penzias & Wilson, 1965).
The Era of Recombination
Before recombination, the universe was a hot, dense plasma composed of protons, electrons, and photons. In this environment, photons were constantly interacting with free electrons through Thomson scattering, preventing light from traveling any significant distance without being absorbed or scattered. This made the early universe opaque, similar to the way fog obscures vision.
As the universe expanded, it cooled, and when the temperature dropped to around 3000 K, protons and electrons began to combine to form neutral hydrogen atoms. This process, known as recombination, drastically reduced the number of free electrons, which in turn decreased the likelihood of photons scattering. As a result, photons were able to travel unimpeded, leading to the decoupling of matter and radiation (Zeldovich et al., 1969).
The Formation of Neutral Atoms
The formation of neutral atoms during recombination was governed by the balance between the rate of electron-proton collisions and the rate of photon interactions that could ionize hydrogen. At temperatures above 3000 K, photons had enough energy to ionize hydrogen atoms, keeping the universe in a plasma state. However, as the temperature dropped below this threshold, the rate of recombination surpassed the rate of ionization, allowing stable hydrogen atoms to form (Peebles, 1968).
The process of recombination was not instantaneous but occurred over a period of time as the universe continued to cool. The concept of the "recombination epoch" refers to this gradual transition, during which the ionization fraction of hydrogen dropped significantly. By the end of recombination, the universe was composed primarily of neutral hydrogen and helium atoms, with only a small fraction of free electrons remaining.
Decoupling and the Cosmic Microwave Background
The decoupling of matter and radiation occurred in tandem with recombination. As photons no longer interacted frequently with free electrons, they began to travel freely through space. This event led to the release of the Cosmic Microwave Background (CMB), which we observe today as the oldest light in the universe. The CMB provides a direct glimpse into the conditions of the universe at the time of recombination, with slight temperature anisotropies offering clues about the density fluctuations that would eventually give rise to galaxies and large-scale structures (Penzias & Wilson, 1965; Planck Collaboration, 2018).
The nearly uniform temperature of the CMB, at about 2.7 K, is a testament to the homogeneity of the universe during this period, while the tiny variations reveal the seeds of future cosmic structure. Observations by satellites such as the Wilkinson Microwave Anisotropy Probe (WMAP) and the Planck satellite have provided detailed measurements of these anisotropies, significantly enhancing our understanding of the early universe and validating the Big Bang model (Bennett et al., 2003; Planck Collaboration, 2018).
The Significance of Recombination
Recombination is a critical event in the history of the universe because it allowed the universe to transition from an opaque plasma to a transparent state, enabling the formation of cosmic structures. Without recombination, photons would have remained trapped, and the universe would not have cooled in the manner necessary for the formation of stars, galaxies, and eventually planets. The decoupling of photons also means that the CMB acts as a cosmic "fossil," preserving information about the universe's early conditions and providing cosmologists with a wealth of data to study its origins and evolution.
The successful prediction of the CMB and its properties, including its temperature and anisotropies, is one of the greatest triumphs of the Big Bang theory. It serves as a crucial piece of evidence for our current understanding of cosmology and continues to be a focal point for research into the fundamental physics of the early universe (Alpher & Herman, 1949).
With recombination complete and the universe now transparent, the next phase in cosmic evolution involved the transition into the Dark Ages, a period dominated by neutral hydrogen, which we will explore further in the following section.
The Dark Ages and Reionization (380,000 years till 1 billion years)
The Dark Ages and reionization represent crucial epochs in the history of the universe, bridging the interval between the formation of the first neutral atoms during recombination and the emergence of the first luminous structures. This period spans from approximately 380,000 years after the Big Bang, when the universe became transparent, to roughly one billion years after the Big Bang, when the first galaxies and stars reionized the universe. A deep understanding of these phases is essential to elucidating how the universe transitioned from a homogeneous and dark state to one rich in structure, radiation, and complex astrophysical phenomena.
The Dark Ages
The Dark Ages commenced after recombination, when the universe had cooled sufficiently for protons and electrons to combine into neutral hydrogen atoms. During this era, the universe was largely dominated by neutral hydrogen and helium, and in the absence of significant light sources, it remained devoid of any new radiation. Photons from the Cosmic Microwave Background (CMB) could traverse freely, but no new luminous sources existed to illuminate the cosmos. As such, the universe persisted in a state of darkness, lacking the intricate structures observed today.
During the Dark Ages, the universe's temperature steadily declined as it expanded. With no substantial sources of radiation, the neutral hydrogen remained largely undisturbed. However, gravitational collapse began to amplify the subtle density fluctuations imprinted during cosmic inflation. These primordial fluctuations were crucial, providing the scaffolding for future structure formation, which enabled the accumulation of matter that eventually gave rise to stars and galaxies (Bromm & Larson, 2004).
The Formation of the First Stars and Galaxies
The conclusion of the Dark Ages coincided with the formation of the first stars, termed Population III stars. These stars were massive, short-lived, and primarily composed of hydrogen and helium, as heavier elements had not yet been synthesized. Population III stars were notable for their immense masses, potentially hundreds of times greater than that of the Sun, and their high temperatures, making them efficient sources of ultraviolet radiation (Bromm & Yoshida, 2011).
The formation of these early stars initiated a transformative phase in the universe's evolution known as cosmic reionization. The ultraviolet radiation emitted by Population III stars began to ionize the surrounding hydrogen gas, dissociating atoms into protons and electrons. This process created expanding ionized regions, or "bubbles," around individual stars and star clusters, which gradually grew and coalesced over time (Fan, Carilli, & Keating, 2006).
In addition to Population III stars, early galaxies and black holes contributed significantly to reionization. As gravitational collapse proceeded, the first galaxies began to form, introducing further ultraviolet radiation into the universe. Black holes, originating from the remnants of massive Population III stars, also played a critical role by emitting high-energy X-rays, which contributed to hydrogen ionization on larger scales (Loeb & Furlanetto, 2013).
The Epoch of Reionization
The Epoch of Reionization (EoR) denotes the period during which the intergalactic medium (IGM)—the space between galaxies—underwent a transformation from a neutral to an ionized state. This transition did not occur uniformly but instead progressed in a patchy manner, as regions surrounding early stars and galaxies became ionized, forming a complex tapestry of ionized and neutral hydrogen.
Reionization began approximately 150 million years after the Big Bang and was largely completed by around one billion years after the Big Bang. The process of reionization fundamentally altered the universe, as it allowed photons from nascent stars and galaxies to travel freely across vast cosmic distances, ending the Dark Ages and rendering the universe transparent to ultraviolet radiation for the first time since recombination.
Observational evidence of reionization is derived from multiple sources. One key piece of evidence is the Gunn-Peterson trough, observed in the spectra of distant quasars. When light from a quasar traverses neutral hydrogen, it is absorbed at specific wavelengths, producing a trough in the observed spectrum. The absence of such absorption in quasars observed at later epochs indicates that reionization was complete by that time (Gunn & Peterson, 1965).
Another vital source of information is the CMB. Measurements from the Wilkinson Microwave Anisotropy Probe (WMAP) and the Planck satellite have provided valuable insights into the timing of reionization by detecting the polarization of CMB photons scattered by free electrons produced during reionization. These observations suggest that reionization was a protracted process, commencing as early as 150 million years after the Big Bang and continuing for several hundred million years (Planck Collaboration, 2018).
The Role of Reionization in Cosmic Evolution
Reionization had profound implications for the universe's evolution. By ionizing the intergalactic medium, it suppressed subsequent star formation in some low-density regions, as the elevated temperature of the gas inhibited gravitational collapse. This regulation of star formation influenced the growth and distribution of galaxies, shaping the large-scale structure of the cosmos.
Moreover, reionization played a critical role in determining the thermal history of the universe. The ultraviolet and X-ray radiation emitted during this epoch not only ionized hydrogen but also deposited substantial energy into the intergalactic medium, heating it to temperatures of several thousand degrees Kelvin. This heating had lasting effects on the formation of galaxies and clusters by influencing the conditions necessary for gas to cool and collapse to form new stars (McQuinn, 2016).
Future Perspectives
The Dark Ages and the Epoch of Reionization represent essential chapters in the cosmic narrative, marking the universe's evolution from a simple, dark state to one teeming with luminous structures and complex activity. Although considerable progress has been made, numerous questions remain unanswered. For instance, the precise nature of the first luminous sources, the detailed chronology of reionization, and the mechanisms by which ionized regions expanded and merged are still subjects of active investigation.
Future observatories, such as the James Webb Space Telescope (JWST), the Square Kilometre Array (SKA), and forthcoming 21-cm experiments, promise to illuminate these enigmatic periods further. These instruments will enable astronomers to explore the formation of the first stars and galaxies, map the evolution of reionization, and provide a more detailed understanding of how the universe transitioned from darkness to the light-filled cosmos we observe today.
Structure Formation
As the universe evolved, gravitational instability amplified density perturbations, causing matter to coalesce into the first galaxies and stars. Dark matter played a crucial role in this process, as its gravitational influence helped pull together ordinary matter, thereby accelerating the formation of galaxies and clusters (White & Rees, 1978). The universe ultimately evolved into a vast cosmic web, characterized by galaxies arranged in filaments, clusters, and voids—a structure shaped by the interplay between dark matter and baryonic matter.
The Modern Universe
Observations of distant supernovae indicate that the expansion of the universe is accelerating, driven by a mysterious force termed dark energy (Riess et al., 1998; Perlmutter et al., 1999). Dark energy is believed to constitute approximately 68% of the universe's total energy content, yet its nature remains one of the most profound mysteries in cosmology.
Galaxies have undergone significant evolution over billions of years, influenced by processes such as star formation, supernova feedback, and galactic mergers. Star formation rates have gradually declined, and many galaxies are transitioning from active star formation to more quiescent states.
Together, dark matter and dark energy constitute approximately 95% of the universe's total mass-energy budget. While dark matter plays a pivotal role in the formation and evolution of cosmic structures, dark energy governs the large-scale dynamics of cosmic expansion.
Summary and Future Perspectives
The evolution of the universe, from pre-inflationary conditions to its current state, comprises a sequence of transformative epochs that have defined its structure and composition. Despite significant advances in our understanding, many fundamental questions remain: What preceded cosmic inflation? What is the true nature of dark matter and dark energy? Upcoming missions, such as the James Webb Space Telescope, Euclid, and the Vera C. Rubin Observatory, hold the promise of addressing these questions, offering new insights into the universe's grand narrative.
References
- Alpher, R. A., Bethe, H., & Gamow, G. (1948). The Origin of Chemical Elements. Physical Review, 73(7), 803-804.
- Alpher, R. A., & Herman, R. (1949). Evolution of the Universe. Nature, 162(4124), 774-775.
- Baumann, D. (2009). TASI Lectures on Inflation. arXiv preprint arXiv:0907.5424.
- Bassett, B. A., Tsujikawa, S., & Wands, D. (2006). Inflation dynamics and reheating. Reviews of Modern Physics, 78(2), 537-589.
- Bennett, C. L., et al. (2003). First-Year Wilkinson Microwave Anisotropy Probe (WMAP) Observations: Preliminary Maps and Basic Results. The Astrophysical Journal Supplement Series, 148(1), 1-27.
- Bromm, V., & Larson, R. B. (2004). The First Stars. Annual Review of Astronomy and Astrophysics, 42, 79-118.
- Bromm, V., & Yoshida, N. (2011). The First Galaxies. Annual Review of Astronomy and Astrophysics, 49, 373-407.
- Fan, X., Carilli, C. L., & Keating, B. (2006). Observational Constraints on Cosmic Reionization. Annual Review of Astronomy and Astrophysics, 44, 415-462.
- Garcia-Bellido, J., Kofman, L., & Linde, A. (1998). Preheating in hybrid inflation. Physical Review D, 57(12), 6050-6060.
- Gross, D. J., Wilczek, F., & Politzer, H. D. (1973). Asymptotically Free Gauge Theories. Physical Review Letters, 30(26), 1343-1346.
- Gunn, J. E., & Peterson, B. A. (1965). On the Density of Neutral Hydrogen in Intergalactic Space. The Astrophysical Journal, 142, 1633-1641.
- Guth, A. H. (1981). Inflationary Universe: A Possible Solution to the Horizon and Flatness Problems. Physical Review D, 23(2), 347-356.
- Higgs, P. W. (1964). Broken symmetries and the masses of gauge bosons. Physical Review Letters, 13(16), 508-509.
- Kofman, L., Linde, A., & Starobinsky, A. A. (1997). Reheating after Inflation. Physical Review Letters, 73(24), 3195-3198.
- Linde, A. D. (1982). A New Inflationary Universe Scenario: A Possible Solution of the Horizon, Flatness, Homogeneity, Isotropy, and Primordial Monopole Problems. Physics Letters B, 108(6), 389-393.
- Loeb, A., & Furlanetto, S. R. (2013). The First Galaxies in the Universe. Princeton University Press.
- McQuinn, M. (2016). The Cosmology of Reionization. Annual Review of Astronomy and Astrophysics, 54, 313-362.
- Mukhanov, V. (2005). Physical Foundations of Cosmology. Cambridge University Press.
- Peebles, P. J. E. (1966). Primordial Helium Abundance and the Primordial Fireball. Physical Review Letters, 16(11), 410-413.
- Peebles, P. J. E. (1968). Recombination of the Primeval Plasma. The Astrophysical Journal, 153, 1.
- Penzias, A. A., & Wilson, R. W. (1965). A Measurement of Excess Antenna Temperature at 4080 Mc/s. The Astrophysical Journal, 142, 419-421.
- Perlmutter, S., et al. (1999). Measurements of Omega and Lambda from 42 High-Redshift Supernovae. The Astrophysical Journal, 517(2), 565-586.
- Planck Collaboration. (2018). Planck 2018 Results. VI. Cosmological Parameters. Astronomy & Astrophysics, 641, A6.
- Riess, A. G., et al. (1998). Observational Evidence from Supernovae for an Accelerating Universe and a Cosmological Constant. The Astronomical Journal, 116(3), 1009-1038.
- Sakharov, A. D. (1967). Violation of CP invariance, C asymmetry, and baryon asymmetry of the universe. Soviet Physics Uspekhi, 34(5), 392-393.
- Steinhardt, P. J., & Turok, N. (2002). A Cyclic Model of the Universe. Science, 296(5572), 1436-1439.
- Vilenkin, A. (1983). Birth of Inflationary Universes. Physical Review D, 27(12), 2848-2855.
- White, S. D. M., & Rees, M. J. (1978). Core Condensation in Heavy Halos: A Two-Stage Theory for Galaxy Formation and Clustering. Monthly Notices of the Royal Astronomical Society, 183(3), 341-358.
- Zeldovich, Y. B., Kurt, V. G., & Sunyaev, R. A. (1969). Recombination of Hydrogen in the Hot Model of the Universe. Journal of Experimental and Theoretical Physics, 28, 146.
Author
Lander Compton
Creation Date
19:11 at 10/25/2024
Last Updated
04:11 at 11/02/2024